17. Key Events in Evolution
EEB 122: Principles of Evolution, Ecology and Behavior?

17. Key Events in Evolution

https://oyc.yale.edu/ecology-and-evolutionary-biology/eeb-122/lecture-17

We've had three lectures about macroevolutionary principles: speciation; how to build a tree; and then what kinds of uses you can put a phylogenetic tree to. Now we're going to do three lectures on different ways to look at the history of life. The first lecture?is an abstract conceptual view of the history of life.?We'll talk about what life is, how we think it originated, the origin of cells and eukaryotes, some other key events; and then we'll summarize with the principles involved in key events, in major transitions in evolution.?
Life is basically anything that has the properties of multiplication, variation in heredity, plus metabolism.?
It's not necessarily carbon-based or silicon-based or based on anything else; it has these abstract properties. And what we really mean by metabolism is something about thermodynamics.
A living thing that has metabolism is using free energy that it's getting from the environment, and it's using that to maintain a partially isolated system?or?a local system. It's preserving the information that evolution and development have given and it's doing so against an entropy gradient. We eat energy and excrete entropy.

Our problem is to figure out how did this all get going? The first major transition was the transition from an abiotic world to a living world; the origin of life. And to do that we had to get multiplication, variation, heredity and metabolism. It is likely that metabolism came first, and that heredity and multiplication came second; variation was probably there from the start.?So I'm going to actually dwell on some things that are now chemically fairly trivial, and some things that are logically non-trivial and involve paradoxes.
The first thing is the environment at the origin. The Big Bang is now dated at about 13.5 billion years. In stars, the nuclei of all of the elements were synthesized out of hydrogen?and some of the novae and supernovae created things that are heavier than iron; and a lot of that is important cofactors for enzymes in your body.
Now the solar system is formed basically from recycled star stuff, about 5 to 4.5 billion years ago, and there's intense planetoid bombardment of the inner planets going on at that time. Nothing living could have existed on the face of the earth, because temperatures were simply too high. The surface of the planet was really a toxic wasteland of boiling lava and very, very extreme temperatures and pHs.?
The moon was formed, possibly because of the impact of a large planetoid that hit the forming earth and actually blew a chunk of it off.?At that point the earth had short days; it was spinning very rapidly. It had condensed from a cloud of stuff, and as it fell in, it brought angular momentum into the system. And so the earth started off with a higher rotation speed than it currently has.
Then about a billion years after the planet forms, the atmosphere is reducing, the temperature has fallen to where liquid water can exist on the surface of the planet. And life probably originates on a positively charged mineral surface, and it seems now likely that it was probably at a deep hydrothermal vent.?There was a hot high-pressure acid around a positively charged mineral surface. There was a lot of chemical energy at that interface. This does seem to be the kind of a context in which you can get certain chemical reactions to work.
You can start with fairly simple things, and natural selection will refine them fairly rapidly, but you have to start with at least these simple things. So that's kind of a bootstrap operation. You've got to get those first replicators up and running before natural selection will take over and create complexity and precision and make it into a sophisticated system.?
You need the building blocks of life. You need linear lipids; they can make membranes and they can make compartments. You need amino acids to make proteins. You need purines and pyrimidines to make nucleotides. You need sugars and phosphates to link things together.
And then after you get the building blocks?and get them to work together, you need things that will make copies of themselves. So if you can get reproduction, variation in reproductive success, some kind of inheritance, then natural selection will take over.
If you can get some kind of primitive genetic molecule, it can get honed into an RNA or a DNA structure. If you can get some kind of primitive cell, with a very simple membrane, then natural selection can hone it into a selective filter, where there are pores in the cell that let certain things in and out, and so forth.?
How do you get the building blocks? Stanley Miller,?working with Harold Urey,?put methane, nitrogen, ammonia, ammonia chloride;?he also?put in carbon monoxide, nitrogen, hydrogen, different kinds of mixtures, into a vessel, and then he would pass an electric spark through it. And he found that it was fairly easy to get these amino acids to fall out.?
The basic structure is that you make something out of fairly small, simple molecules, likely to be in the atmosphere or in the water, on earth,?3.5 to 4 billion years ago. And you give it some energy in the form of an electric charge, or you heat it.
We get amino acids, fatty acids, sugars and purines pretty easily. Pyrimadine nucleotides; ribose is not so easy. A linear, long-chain, fatty acid, that you might find really useful for making a membrane, that's not so easy.?
But once we've got the building blocks, you run into ?an abstract logical problem instead of a?chemical synthesis one.?That's the error threshold.?
The amount of information that you can maintain by selection is limited by copying fidelity. If you have a very high mutation rate, you are destroying all of the information that you've accumulated, and you're not transmitting enough to the next generation to be able to usefully accumulate information on how to do something better.
A small genome is small enough so that the probability of a mutation at any one place in it is fairly small. A big genome is extremely likely to have a bunch of mutations. So there's a relationship between the size of the genome and the amount of mutation that can be tolerated.
So Manfred Eigen claimed that?the relevant mutation rate is non-enzymatic replication. We're going to make?copies of molecules, and at the origin there aren't any enzymes anymore.?Enzymes are things that are produced by living systems; we don't have them. We have to imagine ourselves back into the situation where we don't have enzymes but we want replication.
The maximum genome size is about 100 nucleotides. So when dealing?with replication of a DNA-like or an RNA-like molecule, you can only get up to a genome of about 30 amino acids with the kinds of mutation rates you get with non-enzymatic replication.?

You cannot?get an enzyme out of 100?necleotides, but?1000 to 10,000 nucleotides?to construct any of the things?that we know of now that can do that.?Anything bigger than that, you need enzyme?to make the replication more accurate.?You need translating machinery to take the genome and make it into that enzyme; you need the enzyme then to be working on making the genome replicate accurately. So you can't get enzymes without a big genome, and you can't get a big genome without enzymes. It's a catch-22 paradox.?
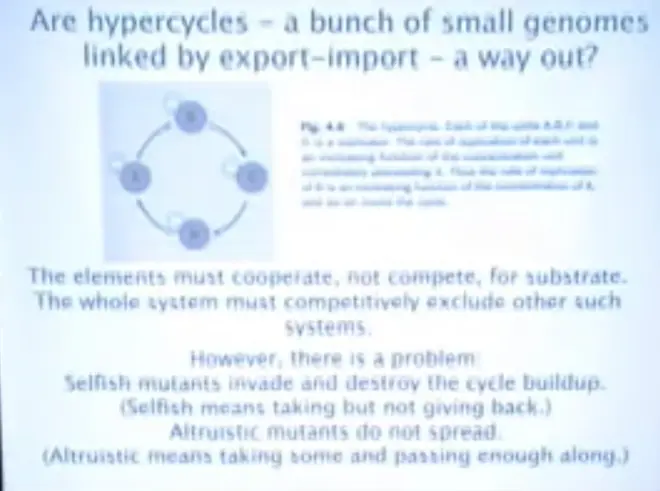
Eigen?introduced?hypercycles to solve catch-22.?It's a chemical reaction that can make more of itself.?It's called a hypercycle because it's a cycle with cycles inside of it. The rate at which any one of these can replicate increases with the amount of product that's being given to it by any of the others. And that means that B can make more B if it's getting more from A; C can make more C if it's getting more from B.
In order to function, the elements have to cooperate for substrate rather than to?compete. They have to take what they need and pass some?on. If this is then going to get refined and become more sophisticated, it might?acquire?an information molecule like DNA,?competitively excluding?other such systems.?
If this is ever going to bootstrap life and get underway, then it has to be isolated in a compartment and start competing with other such systems, because only then will natural selection kick in. It's an open system.?So a?selfish mutant could invade and destroy it. Somebody could come in, who was say an alternate version of C, and take stuff from B and make a lot more C, but not ship anything on to D; it could make a lot more C.?And in this system here, an altruistic mutant won't spread.
If we could get them to be sufficiently complex, then they would start competing with each other, and then?at a certain point in the complexity, they might invent genes and have a competitive upper hand by then being able to transmit the information on what was working.
And they would solve Eigen's paradox?because each one has a small genome, but in sum they would add up to the information in a big genome. So if you could somehow stabilize these small competitive systems so that they had internal cooperation but they were competing between themselves.
So one way out of this problem of the invasion of mutants is to actually put them into compartments.?So you actually have to get the chemical reaction system isolated inside something like a membrane; and that means it's a local group.?
In order to get this going, in order to get the whole process going, basically what you need to do is isolate one of those hypercycles, let it grow, have some kind of division going on, with some inheritance, and then have a mutation that's going to improve one of the descendents.?
Once you get that process going, then you're going to retain cooperation within compartments, you're going to increase competition between compartments, and natural selection can take off and start to produce the very early primitive cells.?
It's associating cause with effect strongly.?If it's open system, you have no guarantee it's going to come back to help you. So there's no guarantee of reciprocity. But if you can contain the cycle, then reciprocity is going to occur because the cycle is shielded from the outside.
It means that all of the elements in the cycle have a common stakein the success of the compartment. They are all in the same boat; they're all in it together. And?they will improve their competition with the other similar things on the outside.?
We don't know where the long-chain fatty acids came from. Let's assume?we have our long-chain fatty acids; and, in fact, we have a special kind. They've got a hydrophilic end and a hydrophobic end. So one end likes water and one end hates water. You have an aqueous solution.?
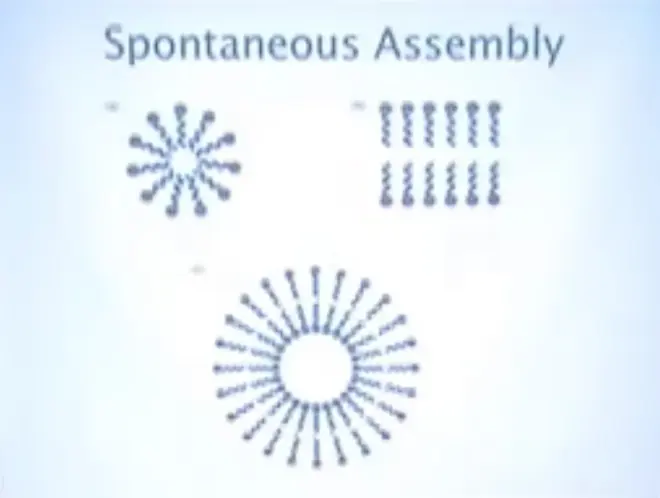
These are things which are either micelles or primitive little spheroids or sometimes linear things, that actually start looking pretty much like a biological membrane. The fatty tails are sticking in and associating with each other, and the hydrophilic parts are sticking out and associating with all of those nice charged water molecules.
Make a mixture of those things, and then put in a surface--so you have either the water surface or a?mineral surface--and this is what naturally happens. This is called abstriction; you will get a little bump forming.
So they will grow and they'll actually kind of start pulsing around a little bit, just from Brownian motion. And if you get these little spheroids, that are lipid bilayers, up to a certain size they will actually divide.
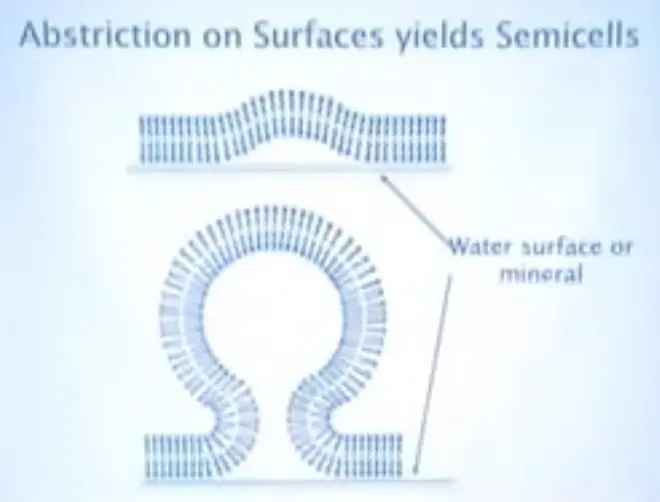
So you can see that it might not actually have been necessary for natural selection to arrange the first replication events for cellular compartments. That might have been something that was just happening physically and chemically, so that part of the bootstrapping was there for free.
If you want to make it grow, then one of the things you want to put inside it is a hypercycle that will make more membrane building blocks.?So perhaps that was actually the kind of chemical reaction that was going on in some of the early semi-cells or proto-cells; they were making the building blocks of lipid bilayers.?
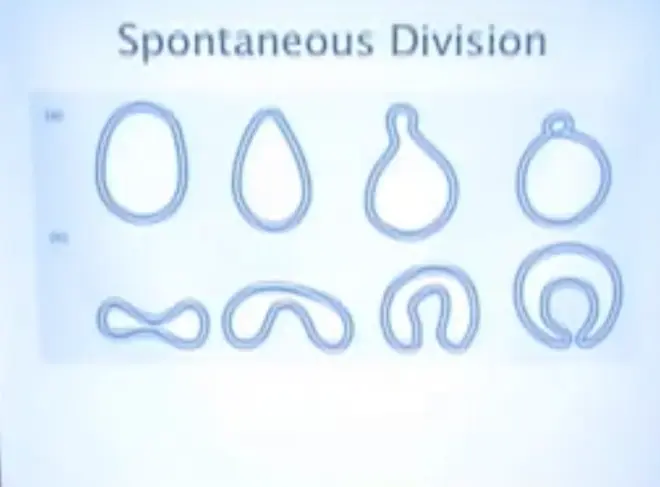
You need?membrane?to isolate?your reactions?so that they can be cooperative and?not de-stabilized by parasites. But then?you have?a problem of how to get the different substrates that you need in through the membrane; that's the problem of membrane transport.?So there's got to be a period, back at the origin of life, during which membrane properties can evolve, and that in a replicating entity that's not yet fully cut off from its environment.?
One possible solution is a semi-cell. That would be you make a cup on the surface of water or?a mineral, and you don't completely close the perimeter. So the thing's kind of leaky; stuff can go in and out of the edges of the cup. It allows a partial openness and?gives you a certain degree of control over who comes in and out, and that might've helped to adjust these early semi-cells.?

We're now going to discuss the prokaryotic/eukaryotic transition. And the prokaryotic/eukaryotic transition is thought to be a major transition because it contains a significant change in the transmission of information.?
The transition?is from an organism, like a bacterium, that has a single circular chromosome anchored to a cell wall, to an organism that has a nucleus within which the chromosomes are?and?there are then often multiple chromosomes inside the nucleus, plus organelles that are out in the cytoplasm, plus a lot of cell structure that's simply not present in the prokaryotes.
One of the things that you have is an organizing center, out of which actin filaments will grow, and these are used in meiosis and mitosis. And associated with that organizing center is a little circular chromosome which is actually thought to indicate that this was originally an independent bacterium, and that these actin filaments are evolutionary homologous with a bacterial flagellum.
You have got vacuoles?and?endoplasmic reticulum. And all eukaryotes, if they have a cilium, they have a characteristic 9 + 2 structure?in the cilium, and this is thought to have been constructed from multiple bacterial precursors.
In the eukaryotes there's an internal cytoskeleton. There are several linear chromosomes?inside a nuclear envelope; transcription and translation are separated; and there are organelles. And, with eukaryotes, you get meiosis. So you get a precise and organized way of making genetically different offspring in each generation.?
So the?prokaryotes?have a?rigid outer cell wall, they have a?circular chromosome?attached to the cell wall; and?the?transcribed mRNA is translated?directly.?The mRNA coming off of the DNA helix, and?little ribosomes?chunking?out protein, and?the?process is being done at?the endoplasmic reticulum, and it's?not direct, it's?indirect.
In order to go from prokaryote to eukaryote, you got to lose your cell wall. And that's a real issue?because the cell wall, in a bacterium, is protecting it from swelling up and bursting like a balloon?due to osmotic pressure. So the bacterium is using a rigid cell wall to protect itself from the influx of water.
And in order to do that, in order to lose the cell wall, you need something to stabilize it on the inside, and that's the cytoskeleton. So the cytoskeleton is really an extremely important morphological invention in this transition.
If you put your DNA into a nucleus, and you no longer have it as a single circular chromosome, then it is possible that without having to attach it to a cell wall, it might make it easier to make multiple chromosomes.?
You get a big advantage from that in terms of how big a genome you can have, because you can then start replication in parallel?at multiple points, rather than going around a single circular chromosome to do it.?And that means that there really would be no upper limit on genome size.
If it's taking an interaction between two or more genes to produce either an organism or to produce a biochemical reaction system that has a certain ratio, that is well balanced in terms of product, then linking the genes that are involved in that ensures they'll both be found in all offspring, and that's a benefit of?a bigger chromosome.
Now once you make chromosomes and you synchronize their replication, then essentially what you've done is you have eliminated the competition of the genes.?
By putting the genes onto a chromosome, you've put them all into the same boat rather than?a situation where one gene might be replicating at a higher rate than another gene, resulting in?unbalanced biochemistry and unbalanced development.
If you've got a primordial cell that has a lot of reactions going on in it, that are being controlled by genes that are all on separate chromosomes, and if those chromosomes are not being divided evenly at mitosis and meiosis, then it's possible that all those reaction systems are going to get out of balance.
And?if you've got unlinked genes with products that need a certain ratio, then getting them onto the same chromosome and regulating them all together, gives you a big advantage over your competitors, at that point.?
Now the other thing that's going on in the eukaryotes is they've got these symbiotic organelles that were originally independent bacteria: the mitochondria, the chloroplasts, and possibly the spindle apparatus.?
One thing that we notice when we look at the chloroplasts in the mitochondria is that the chloroplasts have a much bigger genome than do the mitochondria, and the mitochondrial genes have been transferred into the nucleus.
One of the reasons to do that is essentially efficiency. If you put those genes into the nucleus, you're just maintaining two copies of them, rather than thousands of copies out in the population of mitochondria that are living in the cell.
Another?reason?is conflict resolution. Basically you're cutting way down on the opportunity for different variants to exist in mitochondrial processes.?If you're constructing all of the mitochondria out of essential elements that are in your nuclear genome, you've gotten control over them?and you are making them all look the same, rather than mutating and possibly having the option of creating a runaway mitochondrial cancer that would destroy your metabolism.
Now why not put all the genes into the nuclear genome??
One is that the genetic code is actually different in the mitochondria and in eukaryotes and in the nuclear genome and in the chloroplast. So the tRNA and ribosomal RNA genes, the translation machinery had to be retained in the mitochondrion.
Now that explains the mitochondrion, but it doesn't explain the chloroplast. It looks like the chloroplast retains more genes because it has to move macro molecules through more layers of membrane. It's harder to move big molecules through them, and so if you need them to make the chloroplast function, you have to make more of them locally. So that's probably one reason for the retention of more genes in the chloroplast genome than in the mitochondrial genome. ?
Do we think that the organelles were originally slaves of the things that ate them? Was it a relationship that might have been like a farmer and livestock, the way that corals harvest dinoflagellates, that are helping them make their carbonate skeletons??
We can look at where are the tapping proteins made that are inserted into the organelle membranes? And those are encoded in the nucleus.?And it looks like they evolve from host proteins. So it looks like there have been manipulative steps where the host was actually tapping in to the symbiotic organelles.
They may have been mutualists, and the excretory products of one of them, a eubacterium, was making hydrogen and CO2. That would then have been food for the archaean ancestor of the eukaryotic nucleus. So there is a slave hypothesis and there's a mutualistic hypothesis, and that hasn't been settled.?
Why do plants need to have two? And if you look at their ancestors, you can see that purple non-sulfur bacteria and cyanobacteria--so those are the ancestors of mitochondria and chloroplasts--they can do both; they can both photosynthesize and they can respire.?
The purple bacteria can't photosynthesize when there's oxygen, and the cyanobacteria use the same machinery for both functions, and so that means that the host cell would not be able to separately control photosynthesis and respiration; which one might want to uncouple. And so it appears that mitochondria lost photosynthesis and chloroplasts lost respiration?in coming into the cell.
The world record for membranes around a chloroplast is 4; it's in a dinoflagellate. One thing eats cyanobacterium, and that makes a chloroplast that has the outer membrane of a cyanobacterium and the outer membrane of the cell that ate it, wrapped around it; so that makes two. And then it happens two more times.
It turns out that the things that got eaten to make dinoflagellates had separated, so that there were different kind of chloroplasts that got eaten by different things, that both ended up making dinoflagellates. In that sense, dinoflagellates might be polyphyletic. It's a complicated sequence here.

There are some other key events: origin of the genetic code; origin of multicellularity; origin of germline and soma; origin of social groups; origin of language. There are certain similarities of the process in each one of those steps.?
There is a new level of selection and?replication; the hierarchy is growing. So you go from the hypercycle into competing groups of protocells, and those eventually make a prokaryote.
Then there are symbiotic events, and you actually then have selection operating on a eukaryotic cell with?two or three genomes in it. And then in the process the genetic material?is also going through its reorganization into chromosomes. And then?you get multicellularity; you get cells that are probably genetically almost identical, that are growing together; you get division of labor?and?functional?specialization?within that.
There is a change in the system of information transmission. When you go from prokaryotes to eukaryotes, you have to arrange for information to be transmitted, not only in the nuclear genome, but also in the cytoplasmic genomes. And then you have the evolution of meiosis, and you have sexual reproduction; which is a huge change in the way information is transmitted.
Probably since the origin of sex, the highest impact change in information transmission has been the origin of language and culture, which gives a method of transmitting information from generation to generation, that is independent of the DNA, and allows the process to be going on in different directions at two levels.
In the process of forming this higher level of replication, you have a bunch of lower level units that are coming together to make a higher level unit, and they need to cooperate?properly, but they could be invaded by selfish mutants and?de-stabilized, and so there's a conflict issue. And this conflict is sometimes stabilized by selection for cooperation.?
Because if you are in a tightly spatially organized system, where your own welfare depends directly upon cooperation with another thing in that system, and your system is in competition with some outside system, and the performance of your system is actually a direct function of how cooperative you are, then there is strong selection for integration?within that group. It's thought that this is the kind of thing that's going on when you have a key event in the origin of a new higher level in the evolutionary hierarchy.?
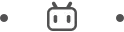