11. Life History Evolution
EEB 122: Principles of Evolution, Ecology and Behavior?

Lecture 11.?Life History Evolution

https://oyc.yale.edu/ecology-and-evolutionary-biology/eeb-122/lecture-11

What life history evolution does is it analyzes the evolution of all of the components of fitness, all the different things that combine to result in lifetime reproductive success, and in so doing it visualizes the design of the organism as an evolutionary solution to an ecological problem. So it's fundamentally about the interface between evolution and ecology, and it is one of the places where scientists confronted the problem of how do we explain phenotypic evolution rather than genetic evolution? So this is really about the design of the large-scale features of organisms.
In the history of ideas,?Darwin showed us that natural selection and descent with modification from ancestors can explain a lot, but then genetics remained a problem until 1900. The role of phenotypes in evolution is the reaction to the neo-Darwinian synthesis.?It has a selectionist part--that is, how are phenotypes designed for reproductive success--and it has a developmental part: what are the restrictions on the expression of genetic variation? So the phenotypes are actually both being designed by natural selection for reproductive success and, in the process of their production, they are themselves editing genetic variation.?
Life history evolution is the part that explains the design of phenotypes for reproductive success, and it concentrates on size at birth, how fast things grow, age and size at maturity, reproductive investment, and mortality rates and lifespan.?

What causes life histories to evolve? They result from the interaction of extrinsic and intrinsic factors.?
The extrinsic factors are things that are influencing the age-specific rates of mortality and reproduction, and that's where ecology?and?phylogenetic effects come in.
There's interaction between extrinsic factors?and intrinsic?factors in?the organism, and the intrinsic factors are conceptualized as tradeoffs among traits. If you change one thing in evolution, a byproduct of that change will be a change in another trait. So even though you are gaining fitness through changes in one trait, almost inevitably, whatever you change is going to cause a decrease in fitness in some other trait, and this forces compromises.
The intrinsic factors are?phylogenetic effects, developmental effects, genetic effects, and?physiological effects.?Tradeoffs in a evolutionary situation are often conceptualized as being strictly energetic. If I take calories away from my growth in order to reproduce and make more babies, then I won't be so big next year to?have so many babies. That would be kind of a standard physiological story about a tradeoff.?But certainly there are developmental and genetic influences on tradeoffs as well.
This standard generic?statement out of life history theory?could be applied to clutch size,?lifespan,?age and size at maturity. They will be optimal when the positive difference between the benefits and the costs?is maximized, for instance,?maturity?at the age and size where the payoff in fitness is going to be greatest.?And we can conceive of that as either being maximized just at a stable equilibrium point?or we can use that kind of analysis to predict a stable equilibrium reaction norm.
Now with--I'm going to show you one way to do this. If we make four general assumptions, we can predict age and size at maturity.
If you're older when you first reproduce, your offspring are going to have better survival rates, they will be of higher quality; so one reason to wait is that you get higher quality offspring.
Another reason to wait is that because you've been growing for longer, you've taken longer to grow before you start to reproduce, you can have more of them?because you're bigger; especially important in plants and in fish.
However, these advantages of delaying maturity are counter-balanced by the advantages of having a shorter generation time, and you can only get a shorter generation time if you mature earlier.
Let me just illustrate the advantage of a shorter generation time.?A shorter generation time is the bank that gives you interest earlier; you get grandchildren quicker.?
So that is basically the elements that you need to put into a quantitative tradeoff. Delaying, you can get higher quality, or more offspring; doing it quicker, you're going to get a shorter generation time and a quicker payoff. Now in a population that's at evolutionary equilibrium, these advantages and disadvantages should have come into balance. So let's see how that might work.?
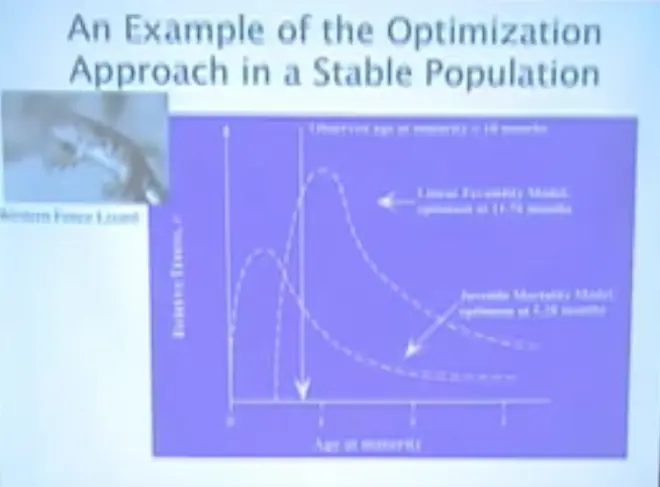
This is using data from the Western Fence Lizard,?where you see these curves going up and down, that's a fitness profile.
In this case it's age of maturity?along the x-axis. Along the y-axis we have relative fitness; so this is the rate at which a population of organisms with that age at maturity would grow, given what we know about the physiology and mortality rates of fence lizards.
If we just put in one of those assumptions, which is that the bigger they are the more babies they have -- so their fecundity grows linearly with size -- their optimal age at maturity is just about twelve months.
If we put in that if they get higher quality offspring as they delay maturity, given the assumptions in the model, we predict actually that they ought to be maturing at about six months. Their observed age at maturity is ten months.?
That indicates that this effect is probably important and perhaps accurately modeled. This number tells us that well perhaps we don't really understand what makes for a good baby lizard. And you can see that interestingly the age at maturity is pretty strongly peaked; the fitness profile has a peak that's pretty close to one value. That means there's pretty strong selection operating on this. It's not flat.?
If you repeat that for a bunch of fish species that are growing in different kinds of conditions--these are haplochromine cichlids in Lake Victoria; the painted greenling lives in Seattle; these roaches are living in Greece. We know growth rates and mortality rates, and we have some estimate of tradeoffs. Then that kind of thinking says this is the predicted age at maturity and this is the observed age of maturity, and the correlation is .93.?
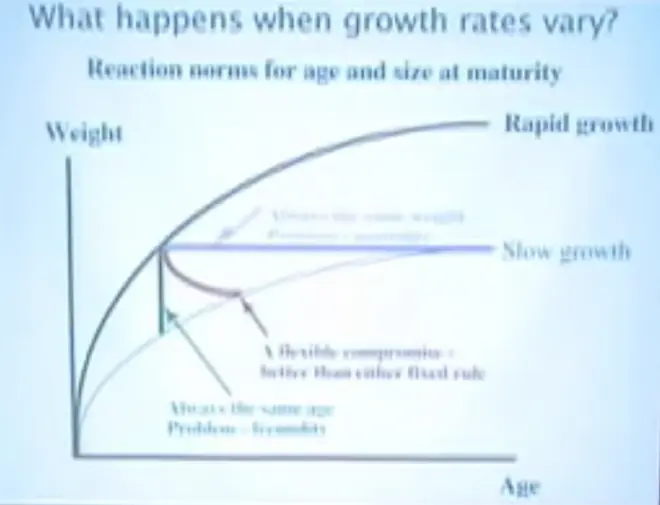
However, that's not the whole story. I now want to extend that to the case when growth rates vary, and I want to introduce you to the idea that age and size at maturity can have a reaction norm.?
Here we have rapid growth. So this is an organism that is born down here, and it's well fed and it grows rapidly. So it gains weight well, reaches a large size.
And this is an organism that grows slowly; it's under food restriction, down here.
Now let's take the blue strategy?and what it says is I'm always going to mature at the same weight. If that organism is growing rapidly, it matures at a pretty early age, but if it's growing slowly and it adheres to this rule, it has to wait a long time until it matures, and its problem here is that it might die before it matures. So that strategy has the cost of mortality.
On the other hand, if it's always the same age when it matures, under good circumstances, it's doing okay, but under poor circumstances it's much smaller, and therefore it can have fewer babies. And so the problem here is fecundity; it's going to not have as many babies if it does that. And so just intuitively you might think that there is some kind of intermediate compromise so that when it is not being fed as much, it changes both its age at maturity and its size at maturity.
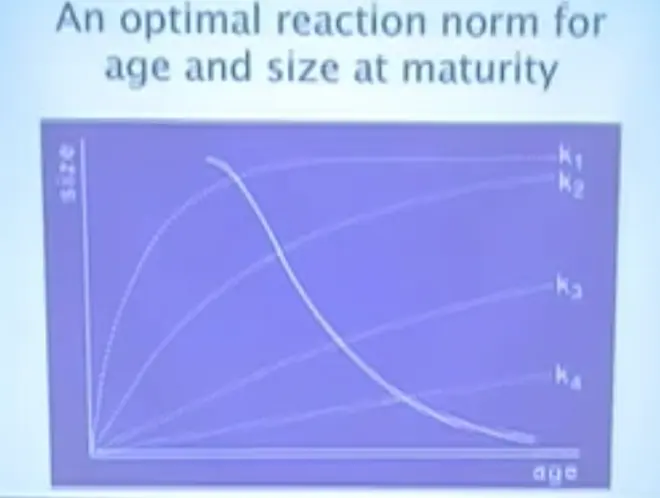
I just want you to take home the message that you can predict what the plastic flexible response should be if evolution has come to equilibrium. And for this one, basically what this graph is telling you is this--this is the reaction norm here, these are growth curves here; so this is good conditions, this is poor conditions--and what this picture is telling is that when life is good, you should mature when you are young and big, and when life is bad, you should mature when you're old and small.
When Nile perch were introduced to Lake Victoria, there hadn't been any Nile perch in there before, and they ate their way around the lake--and in the process?they probably drove about 200 haplochromine species to extinction--but while they going through their initial population burst and they had a lot of food, they were about six feet long. This is the business end of a Nile perch. You can see it's a big fish.
After they had expanded in the lake, which occurred between 1976 and 1979, they ate down the population of their prey, there was not as much food and they didn't grow as well, and they slid down this reaction norm, and now instead of being six feet long, the Nile perch in Lake Victoria are about that big. They still form a fishery and people are still making money on selling Nile perch fillets, but they're much smaller. And that was a predictable thing. And this will happen whenever population densities change.
Back in the 1930s and 1940s, there was a huge sardine fishery off the coast of California. In the 1950s that fishery collapsed, not because of over-fishing, but because of changes in the oceanic conditions where the baby fish were growing up. At the time that it collapsed, there were sardines that had been born under better conditions and started to grow, and then all the competition went away; nobody else came along because all the baby sardines were getting killed by bad conditions out in the ocean.
Just before the fishery folded and there were no longer enough sardines to catch, the fishermen in Monterey were catching female sardines that were one meter long. So they had gone in the other direction, they'd gone up the reaction norm. These things are predictable as population density changes.
I'd like to give you one more example, and it has to do with the issue of whether or not the mammals died out because of bad weather?or over-hunting. Dan Fisher, who's a paleontologist at the University of Michigan, has recovered a lot of mammoth bones from a Native American mammoth slaughterhouse that was outside of Ann Arbor. They used to kill the mammoths and then store them under ice, in a lake, over the winter, so that the other predators wouldn't get the meat. When you look at a mammoth bone, you can tell how big the mammoth was and whether or not it is mature, because the bones of all mammals undergo a change when they reach maturity.
Now if it was bad weather, then they would've been growing slowly, and they should've been small and older when they matured, according to the reaction norm.
If it was hunting, then just like the California sardine, when the population density drops, each individual has more to eat?and they should have been big and young when they matured.
Most people believe the over-kill hypothesis. They were young and large, and some of them had arrow points embedded in their ribs.?
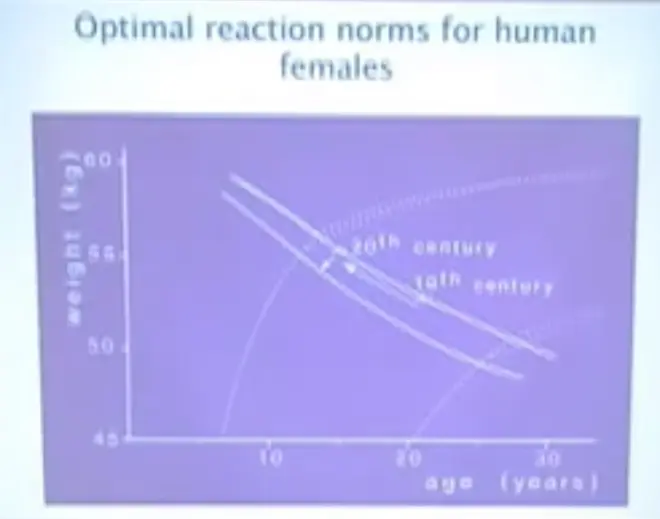
This is what that model tells us about human females. These are some pretty theoretical growth curves for human females under poor conditions and under good conditions. We actually have data on how female age and size at maturity has changed. There are measurements on women working in industrial squalor in North England, in the nineteenth century, and there are good records measured on Hutterite colonies in North America in the twentieth century.?
The nineteenth century women were poorly nourished. The twentieth century women were well nourished. They moved right up a reaction norm. They got younger and bigger when they matured; and it was about four-year advance, earlier maturity in the twentieth century. This is the immediate developmental response to better nutrition.?(the arrow poining up)
If modern medicine were to keep juvenile mortality rates as low as it currently does, then it would cause a further shift in age at maturity in humans.?This probably would take somewhere around 5 or 10,000 years to occur. This is the evolutionary genetic response to a drop in juvenile mortality rates.?(the arrow poining down)

The second major life history trait is once you've matured how many babies should you have, and how big should they be? You want to be an orchid with billions of tiny ones, or you want to be a kiwi with one big one? Well the ideas on this go back to David Lack. David Lack was the man who more or less created the idea of Darwin's finches in the Galapagos.?
If nestling survival decreases as clutch size increases, then an intermediate number of eggs produces the most fledglings.?If you make too many babies, you won't be able to feed them. There are only so many hours in the day. You might be able to work as hard as possible and not bring off a clutch of say ten babies, but you could do quite well with five.
He was wrong on the details, but he got the main point, which is that fitness is often maximized at intermediate reproductive investments, particularly in organisms that reproduce more than once per lifetime. You don't do it all now, you hold some back, and you actually do better if you spread it out.
As clutch size goes up,?eggs go up, but if survival goes down, as eggs go up--this is the per egg survival probability; basically this is saying that if you only laid one egg, you'd have very good survival, and if you lay ten eggs they all die.
What is the equation for how many fledglings for a given number of eggs? It's going to be 1 minus a constant, times the number of eggs you lay, which means, if you multiply that out, that you've got a quadratic term?that means that as clutch size goes up, the number of babies that you get out of it has a parabolic form with an intermediate optimum.?
Then just do the standard basic calculus thing of taking the first derivative, setting it equal to zero. It tells you that this point right here is going to be at 1/2C, in this equation, and if C is 0.1, this optimal number of eggs will be 5, and the number of fledglings that you get out of it will be 2.5. Of course, you never get 2.5, but that's just because the model's continuous and the eggs are discontinuous.?
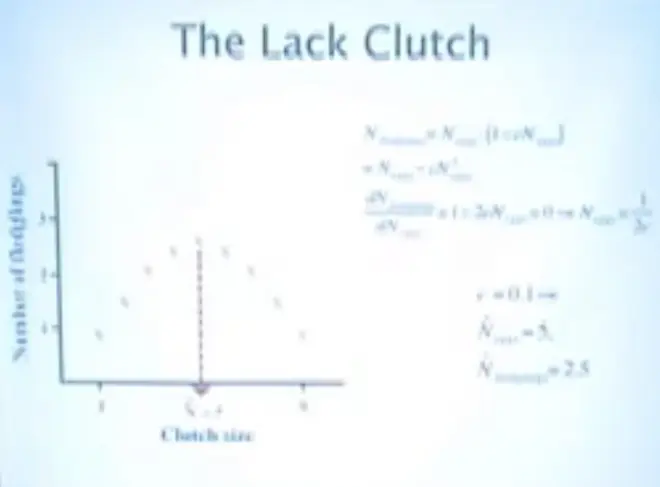
So that?introduces parental survival. If you increase the clutch size, the parents died the next winter at a higher rate, because they worked harder. And if you add all of that up, the residual reproductive value of the rest of their lifetime; the number of grandchildren they would get out of the rest of their lifetime was highest for the reduced broods, intermediate for the control broods, and strikingly lower for the enlarged broods, because of this effect. If you die before the next year, you get zero babies next year.?
So if you look at their total reproductive value, which is the value they got this year, plus the value they got in the rest of their life, it's highest for the control group, and if their clutches were enlarged, they had one grandchild less, and if their clutches were reduced, they had a half a grandchild less. These Dutch kestrels know what's best for them. They lay the right number of eggs. That's the control group.?
Clutch size is trading off with an important fitness component, but it's not fledgling survival, it's parental survival. In this case the reason that they don't lay more eggs is that they themselves are more likely to die; not that their offspring are more likely to die. And these kestrels are optimizing their reproductive investment with a clutch that's of intermediate size.?

Reproductive lifespan?is a balance between selection that increases the number of reproductive events per life--you live longer, you can reproduce more--and effects that increase the intrinsic sources of mortality with age. And it's this idea that there's an evolution of aging or of senescence; there's an evolution of the body falling apart, as a byproduct of something, which is the key feature of this part of life history theory.?
The first kinds of selection pressures are going to lengthen life to give you more reproductive opportunities, but if there are byproducts that are causing intrinsic increases in mortality rate, those will shorten your lifespan. So these things then come into some kind of balance. Any increase in intrinsic mortality rates, or decrease in reproductive rates with age, is called aging or senescence.
Selection operates at different ages. Selection is quite age specific in its impact. Any selection pressure that lengthens life is going to be one that decreases the relative contribution to fitness of offspring, and increases that of adults.
If an adult has survived to some intermediate age, and juvenile mortality in that species is pretty high, then the adult represents a relatively improbable event that's quite valuable, and if it's making babies in that environment, each of them has a relatively low chance of surviving to be that big and that old, and therefore there is a certain fitness advantage in investing in the preservation of that adult, because it's unlikely that you'll get another one up to that state.
The things that will do this are lower adult mortality rates and higher juvenile mortality rates. So if life is relatively good for adults and pretty risky for juveniles?and infants, then you're going to get the evolution of a longer lifespan.
But in contrast, if adult mortality rates increase, then organisms should evolve more rapid aging, basically because there really isn't much point in maintaining a body that's going to be dead anyway for other reasons. Why should I take away from my reproduction and invest it in say disease resistance, or running away from predators, if I'm not going to be able to avoid them anyway
So those are the basic ideas of?why senescence evolves. I'm going to use the fruit fly Drosophila as the model organism. We're going to start this thing off, not when it's an egg, but when it ecloses and is an adult, and we're going to say that our model has no intrinsic mortality at all. So this one doesn't age; this is our baseline, this is what happens if an organism doesn't age.?
Its risk of dying is 20% per day, and every day it lays ten eggs. So on the first day it gets ten eggs. On the second day 80% of them are still around, and each of them lays ten eggs, and on the third day 64% of the original are still around (.8 times .8), ten eggs...?And this thing is potentially immortal. So it can just go on pumping out the eggs, if it survives for as long as ever; and its probability of survival isn't changing with age, it's 80% each time. This one gets 50 progeny. It is calculated by using an infinite series.
Now, what happens if everybody dies between the nineteenth and the twentieth day? That one gets 49.3. That's all the difference that death at old age makes. And this is in a case where there's no senescence.
However, now let's throw in a little life history tradeoff, and it's a really small one. This genotype here, because it can lay eleven instead of ten eggs, on the first day of life, dies, between the nineteenth and the twentieth day, it leaves 50.3 progeny. It has a .6% fitness advantage. If we introduce this genotype into the populations of the ones that live forever, it will take over. There won't be any immortal flies anymore. There will be flies that have evolved a shorter lifespan because they had a reproductive advantage early in life and it didn't take much of one to do it.?
What it shows you is that as soon as you become a teenager and you have some probability of surviving in that human population, your fitness starts to drop, because as soon as you've had a baby, you have some probability of grandchildren. And it shows you that after the age of 46, evolution doesn't care if you're there anymore, from the point of view of getting grandchildren.?
Aging is a byproduct of selection for reproductive performance, and the reason that it occurs is that there's an accumulation of a lot of genes, and they have positive or neutral effects on fitness components early in life, and they have negative effects on fitness components late in life.?
The positive effect is called the antagonistic pleiotropy hypothesis. The idea is that the gene has two effects: good early and bad late. It's like that one that gave the fly one more baby, on the first day of life, but killed it off at the nineteenth day of life.
And neutral effects early and negative effects late is called the mutation accumulation hypothesis.
And these two hypotheses formed sort of the intellectual basis of research on the evolution of aging for quite awhile, and they turn out to be not too productive. In fact, most of the cases that have been well investigated, suggest that it's positive effects early and negative effects late; not neutral early and negative late.?
Organisms age is actually the best evidence?that it's the replication of genes, not the survival of organisms, that is the object of evolution. So that gives you strong empirical evidence that a gene-centered view of evolution is in fact empirically correct. This is extremely discouraging for the organisms that have consciousness and the ability to analyze a situation.?
The result is that after five years, which is about 70 to 110 generations, in these flies, aging evolved as expected. The higher extrinsic mortality rates have produced shorter intrinsic life spans, and the change was about five days. It's convenient that a day in the life of a Drosophila is about a year in the life of a human. So that gives you some feel, some kind of intuitive feel for what this means.
Basically what that means is that if we had started applying this strength of selection at the time of the Trojan War, we would have produced a response in the human population of about five years by now.?

To summarize today's lecture, all the major life history traits--age and size at maturity; number and size of offspring; lifespan; reproductive investment--are involved in tradeoffs, and that causes them to come to evolutionary equilibrium at intermediate, not at extreme values. They are all under stabilizing selection caused by tradeoffs. Age and size at maturity, number of offspring per birth and per lifetime, and lifespan and aging have all evolved.?
I'll just riff on this for a moment, to tell you how you've changed, compared to chimpanzees and bonobos.
Humans live a bit longer, about oh twenty years longer. The unique human life history traits that appear to have evolved since we shared ancestors with chimpanzees and bonobos are menopause, which does occur, but rarely, in zoo chimps, and is almost never observed in the wild.?
The most striking thing though is that we can have babies twice as fast as they can.?The average time in a Neolithic or hunter-gatherer society, between births is two years, in humans, and in chimps it's five to six. That, despite the fact that human babies are much more helpless and need a lot more parental care when they're born. So, in fact, humans have somehow managed to almost double the reproductive output of?chimpanzees, and it appears that they've done it through social interaction. Humans?have a much better integrated family life.?
The evolution of all of these traits can be understood, in general, as an interaction between extrinsic ecological conditions, that determine mortality rates, and conditions inside organisms to cause tradeoffs. So if you're looking for a general explanatory structure, it is that the environment poses problems, and when you answer that problem with a solution, you are forced to make compromises.
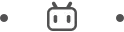